Assist. Prof. Panagiotis Tassis
Assistant Professor of Swine Medicine and Reproduction, Clinic of Farm Animals, School of Veterinary Medicine, Aristotle University of Thessaloníki, Greece
Mycotoxin menace in grains worldwide
Mycotoxins are secondary metabolites produced by fungi (genera Aspergillus, Penicillium, Fusarium, Alternaria, and Claviceps) that can be found in grains (e.g., maize, wheat, barley) worldwide.
for swine health and production2.
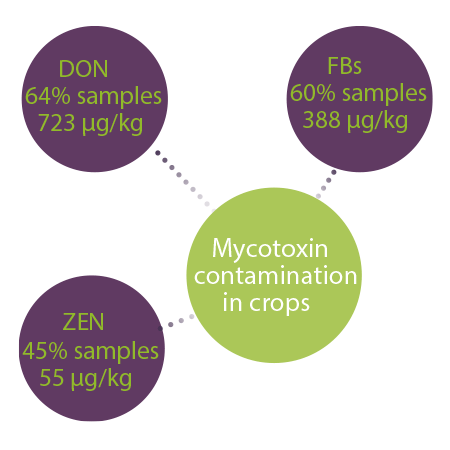
Recent studies have suggested that up to 80% of feed and food crops are contaminated with mycotoxins globally (occurrence above the detectable levels up to 60–80%), whereas co-contamination of grains with multiple mycotoxins is a common finding3.
A 10-year survey with samples from 100 countries reported that DON, FBs, and ZEN were most prevalent mycotoxins and were detected in 64%, 60%, and 45% of all samples, respectively. The median concentrations were 723 μg/ kg, 388 μg/kg and 55 μg/kg for FBs, DON and ZEN, respectively1.
Pigs and poultry are very susceptible and sensitive to the effects of mycotoxins4.
The effects of mycotoxins on pigs are multiple and they depend on5:
- ⇰ The type of mycotoxin
- ⇰ The level and duration of exposure
- ⇰ The age of the animal
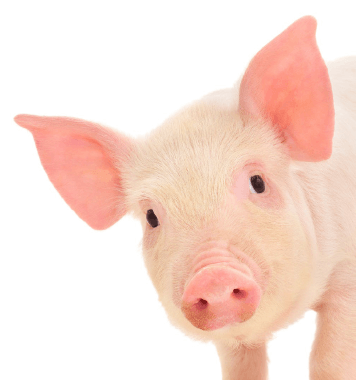
Ingestion of great dosage levels can induce acute cases of mycotoxicosis with well-described clinical symptoms, such aso2,4,6:
Reproductive disorders and hyperestrogenism syndrome in the case of ZEN
Vomiting and growth retardation in the case of DON
Pulmonary edema after FBs ingestion
Reduced feed intake and weight gain in acute AF cases
Polydipsia, polyuria, and reduced growth in OTA cases
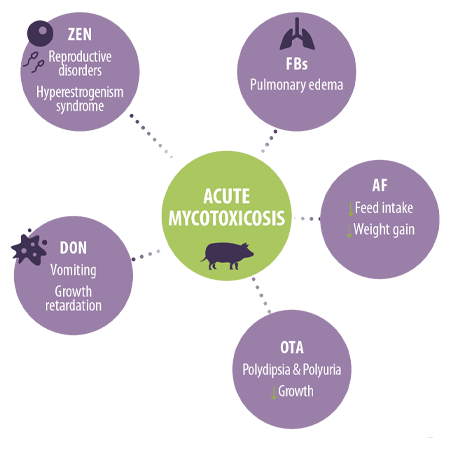
However, chronic consumption of low mycotoxins levels and the induction of vague clinical symptoms seems more probable under field conditions.
The chronic toxic effects of mycotoxins in swine include hepatotoxicity, genotoxicity, nephrotoxicity, neurotoxicity, reprotoxicity, immunotoxicity as well as other effects such as neuroendocrine disorders6,7.
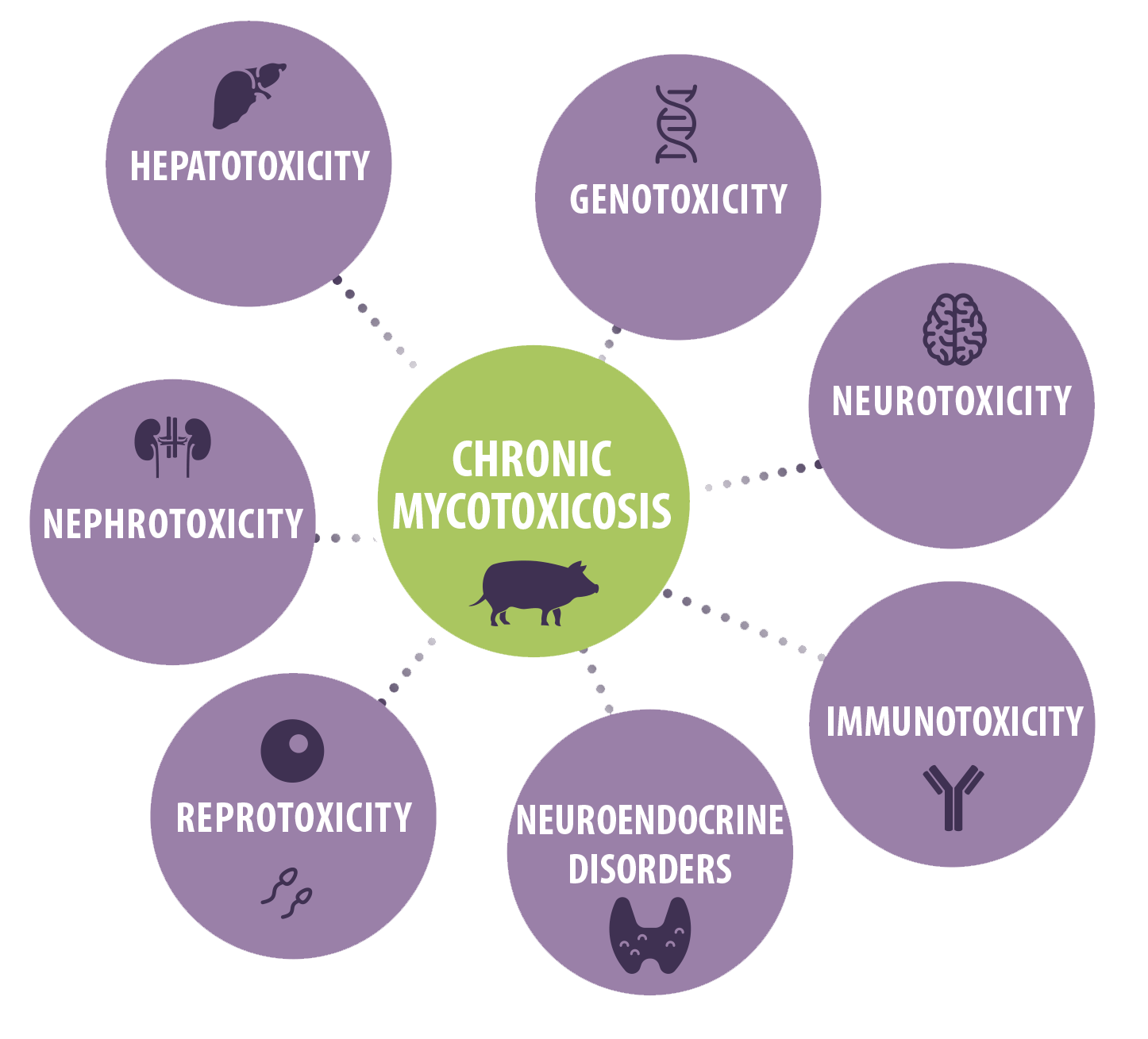